Dynamic Displays in Nature
Dynamic Displays in Nature
Squid, cuttlefish, and octopus developed elaborate skin displays millions of years ago, with performance that is still superior in many ways to today’s leading display technologies. Studying the mechanisms by which animals rapidly and efficiently change their coloration and patterning using only ambient light can offer bio-inspired routes to improved display technologies.
by Lydia M. Mäthger and Roger T. Hanlo
OVER millions of years of evolution, a small subset of animals has developed the ability to change color and pattern for camouflage and communication. The best known are probably chameleons and cephalopods (squid, cuttlefish, and octopus, as shown in Fig. 1) although lesser known examples include certain fish and amphibians. Cephal-opods are by far the most versatile and can change in as little as a quarter of a second. While the majority of animals communicate through various forms of body language, as well as verbal and/or non-verbal (acoustic) signals, cephalopods have surpassed other animals in terms of developing a form of skin “display” that they can use to communicate specific messages. And, of course, their camouflage abilities are legendary.
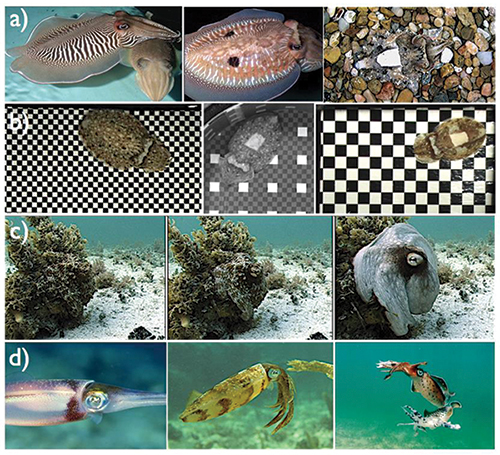
Fig. 1: Examples of skin displays for communication and camouflage in cephalopods include: (a) from left to right, cuttlefish in a zebra pattern, a “deimatic” or threatening pattern with false eye spots, and a camouflaged disruptive pattern; (b) cuttlefish on artificial checkerboard substrates showing high-contrast patterns whose spatial frequencies match those of the backgrounds; (c) an octopus in camouflage showing a rapid transition (a total of 2 sec from left to right) to signaling as the photographer approaches it more closely (in this example, the signal is a threat display, in which the animal makes itself conspicuous with the intention of intimidating a potential predator); and (d) squid showing a “pied” signaling pattern, a camouflaged disruptive pattern, and a fighting pattern. Photographs courtesy R. Hanlon.
Lately, biologists and engineers alike have been particularly interested in the mechanisms underlying color and pattern changes in cephalopods because the structures involved are so effective at creating and mixing multiple colors – all using ambient light. Over the past 2 decades, engineers have been developing and improving various aspects of display technology (e.g., e-Paper, flexible, or conformal displays) with the goal of making devices that can be used in any lighting environment and even in complex geometries like those required for wearable displays. Thus far, in comparison with the color and pattern changes of cephalopods, the display technologies lag behind in optical performance, especially in color generation.1 Furthermore, at best, displays today are flexible, which is still a far-cry from the stretchable/conformal nature of displays in nature. In this article, we describe the optical structures and the physical mechanisms that make cephalopod color and pattern changes possible, with an attempt to identify some areas that engineers working on research and development aspects in the display industry may want to look to for inspiration.
Cephalopod Display “Materials”
In cephalopods, color and patterns are created by the action of millions of chromatophores, which are small pigmented organs (grouped into 2–3 color classes depending on the cephalopod species – red, yellow/orange, and brown/black) and structural reflector cells.2 A cephalopod chromatophore consists of a pigment-containing sac that has dozens of radial muscle cells attached around its periphery [Fig. 2(a)]. The chromatophore muscle cells are controlled directly from the brain (not local stimuli), and through contraction and relaxation of these muscles, the pigment sac increases or decreases in area in less than 1 sec. This unusual mechanism of colorant transposition could be amenable to some materials science applications.
A key feature of adaptive coloration in animals is the refined interplay between pigments and reflectors. In addition to the pigmented chromatophores, cephalopods have iridescent reflectors termed iridophores in many parts of the body [Fig. 2(b)], and these cells have precise arrangements that may perform specific behavioral
functions. (An iridescent structure can be described as having a rainbow-like appearance.) Iridophores are colorless cells that vary in size but are generally smaller than 1 mm.3 They contain stacks of thin plates that reflect light by thin-film interference. Multilayer (Bragg) reflectors have well-defined optical features, the most obvious of which is their angle dependence. The more oblique the angle of incidence, the shorter the peak wavelengths of the reflected light. Furthermore, at around Brewster’s angle, the reflected light is maximally polarized, an interesting property that may have behavioral functions because cephalopods have the ability to see polarized light. It has been speculated that cephalopods could even communicate this way as a form of “hidden” communication channel (since many vertebrates, i.e., potential predators, lack the ability to see polarized light). This “communication” based on polarization may be of interest to display practitioners, especially those working in 3D displays that rely upon polarized light.
It is now known that some squid have the rather sophisticated ability to spectrally tune their iridescence and even switch it off completely. In Loligo squid, some iridescence is shown strongly during fighting encounters between animals and completely switched off at other times. In contrast to chromatophores, which can change within a fraction of a second, iridophore reflectance changes take longer, e.g., several seconds to minutes. In some squid, the reflected wavelengths can shift by over 100 nm, e.g., from infrared to red to orange, but also in other parts of the spectrum. Knowledge of exactly how these wavelength changes are generated is still patchy. The plates of squid iridophores are made up of proteins called reflectins4 One explanation of the observed changes in reflectance is that the protein state changes, affecting refractive index, to first enable the cell to reflect light (this would be the “on/off switch”). Secondly, with higher levels of stimulation (e.g., applying the neurotransmitter acetylcholine that drives these changes), there is a change in the thickness of plates, which causes a spectral change. Essentially, one could consider this as chemically tuned color.
Kramer et al.5 showed that reflectin proteins have remarkable self-assembling properties and that they can be processed into thin films, photonic grating structures, and fibers. Subsequent results show that tyrosine phosphorylation of reflectin proteins is involved in the regulation of iridescence in squid.6 Tao et al.7 studied recombinant reflectin and showed that protein assembly can be triggered by chemically stimulating the cells and that reflectin protein assembly is reversible and tunable.
These properties and operations should provide a valuable background for creating a similar type of system for optical engineering. The applicable value of such a tunable device for the military, etc., may be substantial (see, e.g., a recent paper by Phan et al.8). These shifts of reflectance peaks are directly relevant to display technologies that also shift optical interference, for example, P-Ink by Opalux and mirasol by Qualcomm, and are more loosely related to switchable Bragg reflectors that underpin the cholesteric displays developed by Kent Displays and Fujitsu.
An interactive way in which cephalopods change color is by filtering the iridescence through the pigmented chromatophores [Fig. 2(c) and 2(d)]. This effect can be immediate because chromatophores can be activated in a matter of milliseconds. By selective expansion, chromatophores can either change the reflected spectrum
of the iridescence, create contrast against which iridescence is viewed, or block it altogether. Iridophores can also enhance the chroma-tophores’ appearance. In squid, three classes of chromatophores (yellow, red, and brown pigments), combined with dynamically changeable iridophores, can produce colors that encompass the entire visible spectrum, enabling a wide range of skin colors. It appears that nature has once again perfected an approach that we are just now trying to implement commercially; namely, multi-layered reflective displays that rely on subtractive color such as those in development by Ricoh (electrochromic) and Hewlett-Packard (electrokinetic).
Another type of structural reflector that was originally described only for cuttlefish and octopus is the white-scattering leucophore [Fig. 2(e)]. A study published earlier this year9 shows that leucophores are cells that contain thousands of spherical microparticles called leucosomes that consist of sulfated glycoproteins or proteoglycans and reflectin. The leucophore whiteness is produced by incoherent scattering based upon a randomly ordered system. Leucophores are soft and compliant, and this system may in the future provide an interesting template for approaches to efficient light scattering in materials science and optical engineering. While the cuttlefish system is an entirely passive light diffuser, recently a tunable version has been described in squid.10 Display engineers developing stretchable displays that require reflectors or diffusers may consider the construction and physics that nature has developed for the white-scattering leucophores.
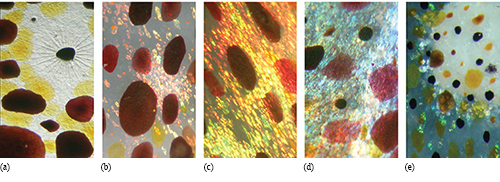
Fig. 2: Chromatophores, iridophores (iridescent reflectors), and leucophores (spherical scatterers) are responsible for color and pattern change in cephalopods. Image (a) shows brown, yellow, and red chromatophores in the squid Doryteuthis pealeii. Located above the center of the image is a partially retracted brown chromatophore, with muscle cells clearly visible. These muscles are responsible for expanding the chromatophore pigment to full size. For scale, a fully expanded squid chromatophore (e.g., bottom right of image a, but also images b, c, and d) measures about 1–2 mm in diameter; a retracted chromatophore (e.g., small dark punctate structure above/left of the large expanded one) measures less than a tenth of a millimeter. In (b), iridophores (the smaller patches of red, pink, yellow, and green) appear underneath the brown and red chromatophores. Shown in (c) and (d) are examples of how chromatophores filter light reflected from iridophores. A red chromatophore at the right side of image (c) can be seen to give the reflected yellow iridescence a red hue. A red chromatophore toward the right of image (d) turns the underlying blue iridescence into purple. A similar effect can be seen in the other color classes of chromatophores. Shown in (e) is a closeup of part of a white fin spot in the cuttlefish Sepia officinalis. The fin spots are made up of leucophores and iridophores. Some iridophores around the periphery can be seen to reflect green light. Above the fin spot are pigmented chromatophores. For scale, the fully expanded chromatophores measure about 1 mm in diameter. Photographs courtesy L Mäthger.
How does the integrated system of pigments and reflectors work? A glance at Fig. 3 may help the display-industry reader appreciate the way these structures
interact with light. The skin of cephalopods is layered so that each of the structures interacts with the incident and/or reflected light in some way. Generally (as with anything in nature, there are some exceptions!), we can say that the pigmented chromatophores are the most superficial structures. Beneath the chromatophores are the structural reflectors such as iridophores, while the white-scattering leucophores are generally found in the deepest layer.
Figure 3 illustrates three sample ray traces (1, 2, 3) incident on the skin. The top panel (a) and the bottom panel (b) are the same, except that the chromatophore diameters are different in each, depicting how the natural system works. Ray trace (1a) shows a ray of white light incident on an iridophore cell. Due to constructive interference, the reflected light is blue, given the periodic spacing of the plates in that iridophore. Ray trace (1b) shows that when the brown chromatophore expands, it absorbs most of the reflected blue light. Ray trace (2a) shows diffusely reflected white light from a leucophore, which in
(2b) is red because it first passes through the expanded red chromatophore. Ray trace (3a) depicts incident light filtered by a yellow chromatophore and
then reflected from an iridophore with a plate spacing shifted closer to the red spectrum such that the reflected light is orange. Ray trace (3b) shows the same yellow-filtered light appearing greenish because the iridophore plate spacing decreased and caused a wavelength shift toward the shorter end of the visible spectrum. These are just a few examples of how chromatophores, iridophores, and leucophores interact to produce a wide range of visual effects.
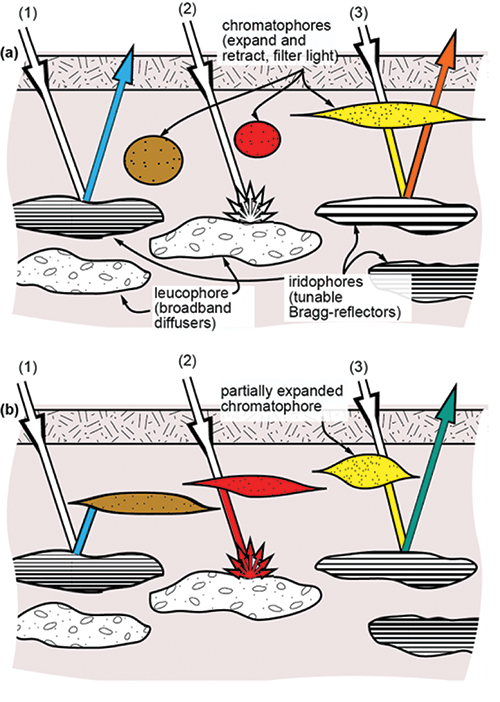
Fig. 3: This diagram of cephalopod skin shows how the three main skin structures – chroma-tophores, iridophores, and leucophores – interact to produce a range of visual effects. Shown are two example states (a,b) and three distinct ray traces (1, 2, 3). After Kreit et al.1
It is interesting to note that these animals are able to “interconnect” all these optical layers, switch them independently, and do so such that their
peripheral interconnects (nerves, supply of nutrients, etc.) have almost no optical loss or influence on the reflected color. Today’s best display technologies are nowhere near this level of optical transparency for interconnects, let alone also making them flexible or conformal/stretchable.
Biophotonic Systems as a Source for Advances in Displays
In terms of the use for active display in nature, cephalopods change skin color and pattern not only for adaptive coloration (i.e., camouflage) but also for communicative purposes, such as to attract mates and deter rivals or to dazzle potential prey and confuse a possible predator. Communicative signals can be elaborate and colorful. Most of the time, they involve high-contrast visual effects (see Fig. 1). For example, the “zebra” display shown by cuttlefish males during courtship is produced by maximally expanding the dark chromatophores in specific areas to achieve a dark striped pattern and by retracting the chromatophores in between, thereby exposing the bright white leucophores underneath. In another example of a high-contrasting pattern, a set of “false eyespots” is displayed. These are intended to startle an approaching predator. Many cephalopod species have dozens of pattern variations to choose from in order to communicate and camouflage effectively in their habitat.
In addition to the colors and patterns created by the skin, various body postures are used to enhance the visual effect, making the adaptive and communicative patterns even more effective. While cephalopods are restricted in the way in which they use their skin structures for camouflage and communication – the patterns are pre-set in specific ways and the system cannot produce an infinite number of patterns – it is certainly possible that this kind of biophotonic system could produce a very large number of patterns if adapted appropriately. Modern displays adjust to their environment typically only in terms of brightness. The algorithms that animals utilize could be exploited by display engineers to find optimal ways to adjust patterns, background intensities, and other factors to maximize the ability to communicate information clearly to the user. Animal adaptive coloration provides excellent inspiration, as animals often select a non-obvious choice of pattern display, which achieves the desired end-result with minimal “display resolution.”
We have seen that there are many similarities in the way that cephalopods have mastered the challenges of creating effective displays and the way that engineers are
seeking to solve the same types of problems. Both use pigments that are spread out or compacted to achieve a visible effect. Cephalopods use muscle fibers; in electronics, electric fields do the trick. However, nature has had a long head start in creating an efficient and sophisticated solution, and there are still things we can learn from studying this natural system. Foremost among these is that cephalopod color and pattern changes make use of ambient light. The animal’s skin reflects, transmits, absorbs, and scatters light in specific ways (some structures even fluoresce) to achieve an optical effect. In this regard, cephalopods are more efficient than electronic devices because the latter generally require electric power to generate emissive light, whereas the structures in cephalopods (once developed) need energy only to enable change – the color production itself requires no energy at all. A natural feature that we may particularly learn from is that in cephalopods the final skin “display” is the result of the optical interaction between a number of different structures (pigmentary and structural). To date, we do not fully understand how these structures interact to produce the final appearance of the animal, but the fact that pigments and structural reflectors are used together (and many animals do this, not just those that can change color and pattern) seems to indicate that there is a fundamental mechanism worth paying attention to.
Another notable feature of cephalopod skin is the flexibility with which the animals can create three dimensionally textured surfaces. Particularly cuttlefish and octopus can selectively wrinkle and fold their skin (the intention of this is to be able to camouflage next to textured objects when the need arises). How these animals accomplish this may be of particular interest to researchers and engineers working on display technologies that require surface texture.
Perhaps it is worth quoting the saying placed over the library entrance door in the 1890s at the Marine Biological Laboratory: “Study nature, not books.” Nature provides a deep repository of elaborate biophotonic systems, and many, if not most, of these remain unexplored. Whether our research is in the generation of color and pattern for displays, textured surfaces, or flexible displays that can be stretched and rolled, cephalopods, fish and some terrestrial color changing animals may still be able to show us a few tricks along the way.
References
1E. Kreit, L. M. Mäthger, R. T. Hanlon, P. B. Dennis, R. R. Naik, E. Forsythe, and J. Heikenfeld, “Biological vs. electronic adaptive coloration: how can one inform the other?,” Journal of the Royal Society Interface doi: 10.1098/rsif.2012.0601 (2012).
2R. T. Hanlon, and J. B. Messenger, Cephalopod Behaviour (Cambridge University Press, Cambridge, MA, 1996).
3L. M. Mäthger, E. J. Denton, N. J. Marshall, and R. T. Hanlon, “Mechanisms and behavioral functions of structural colouration in cephalopods, Journal of the Royal Society Interface 6, S149–S163 (2009).
4W. J. Crookes, L. Ding, Q. L. Huang, J. R. Kimbell, J. Horwitz, and M. J. McFall-Ngai, “Reflectins: The Unusual Proteins of Squid Reflective Tissues,” Science 303, 235–238 (2004).
5R. M. Kramer, W. J. Crookes-Goodson, and R. R. Naik, “The self-organizing properties of squid reflectin protein,” Nature Materials 6, 533–538 (2007).
6M. Izumi, A. Sweeney, D. DeMartini, J. Weaver, M. L. Powers, A. Tao, T. V. Silvas, R. M. Kramer, W. J. Crookes-Goodson, and L. M. Mäthger, “Changes in reflectin protein phosphorylation are associated with dynamic iridescence in squid,” Journal of the Royal Society Interface 2010, 549–560 (2009).
7A. R. Tao, D. G. DeMartini, M. Izumi, A. M. Sweeney, A. L. Holt, and D. E. Morse, “The role of protein assembly in dynamically tunable bio-optical tissues, Biomaterials 31, 793–801 (2010).
8L. Phan, W. G. Walkup, D. D. Ordinario, E. Karshalev, J.-M. Jocson, A. M. Burke, and A. A. Gorodetsky, “Reconfigurable infrared camouflage coatings from a cephalopod protein,” Adv. Mater. DOI: 10.1002/adma. 201301472 (2009).
9L. M. Mäthger, S. L. Senft, M. Gao, S. Karaveli, G. R. R. Bell, R. Zia, A. M. Kuzirian, P. B. Dennis, W. J. Crookes-Goodson, R.
R. Naik et al., “Bright white scattering from protein spheres in color changing, flexible cuttlefish skin, Advanced Functional Materials, 10.1002/adfm.201203705 (2013).
10D. G. DeMartini, A. Goshal, E. Pandolfi, A. T. Weaver, M. Baum, and D. E. Morse, “Dynamic biophotonics: female squid exhibit sexually dimorphic tunable leucophores and iridocytes,” Journal of Experimental Biology 216, 3733–3741 (2013). •
Lydia M Mäthger is Assistant Research Scientist at the Marine Biological Laboratory located in Woods Hole, Massachusetts. She has studied the mechanisms and functions of camouflage and communication in animals, specifically, the physics and biology of coloration and patterning, for 15 years. She can be reached at lmathger@mbl.edu. Roger T. Hanlon is Director of the Program in Sensory Physiology and Behavior at the Marine Biological Laboratory at Woods Hole. He holds professorships at Brown University and Rice University and his laboratory has studied rapid adaptive coloration in marine animals for 30 years.